Maladaptation
In the previous post on The G-CAT, we talked about the role of maladaptation in the evolution of populations and species, and how this might impact their future. To summarise, maladaptation is the process (or trait responsible for) which causes a reduction in the fitness. As we discussed, this can come about a number of ways – such as from a shift in the selective environment or from fitness trade-offs in traits over time – and predominantly impacts on species by reducing their capacity to adapt. Particularly, this is important for small populations or those lacking in genetic diversity, which are already at risk of entering an extinction vortex and lack the capability to respond well to extreme selective changes (such as contemporary climate change).
The role of humanity
Unsurprisingly, human impacts across the globe can have substantial ramifications for the extent and strength of maladaptation. Given the non-specific nature of the mechanisms of maladaptation, this can encompass both ‘natural’ (such as historical climate change) and ‘anthropogenic’ (such as environmental destruction) drivers. While we might struggle to address the former (and indeed the philosophical debate about whether we should seek to change nature), the latter is well within our means (and responsibility!) to tackle. But how do humans drive maladaptation? What factors might cause a population to become maladaptive, or to spread maladaptive traits?
Ecosystem-level impacts
Habitat modification
One clear way human impacts might drive the occurrence of maladaptation is through significant habitat modification. Degradation of habitat from its natural state, or the fragmentation of ‘pristine’ habitat into isolated segments can significantly alter the selective environment under which traits may evolve. With the rate of anthropogenic impact on ecosystems globally, in many situations this can cause shifts in environmental traits faster than native species can adapt to the changes.
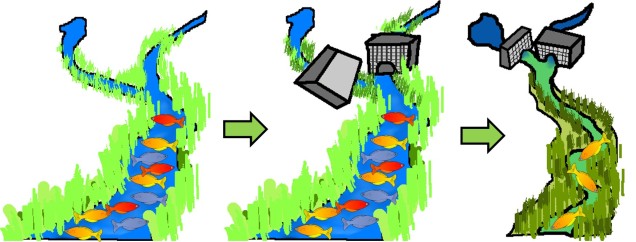
This is represented to a degree by arguably one of the most fundamental demonstrations of Darwinian evolution: the peppered moth. In its unaltered environment, two different forms of the moth can be found: a black coloured variant and a white coloured variant. Given the natural camouflage provided by the white moth colour-matching local trees, its occurrence was much wider spread throughout the population than the black (melanic) variant. However, with the Industrial Revolution across England in the early 1800s, these trees become largely impacted by the outpouring of soot, and the bark darkened substantially. This drove a change in the selective environment as well: rather rapidly, white-coloured moths became less adaptive due to high conspicuity and melanic moths rose in frequency. The rapid change in the frequency of these phenotypes is, to this day, considered one of the most textbook examples of natural selection in the wild (and was driven by human modification of habitat).
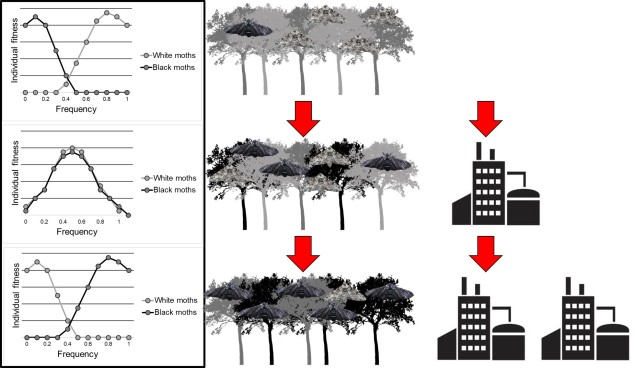
Of course, where there is adaptation there is maladaptation, and within this scenario we could consider industrialisation a driven of maladaptation of the white-coloured phenotype.
‘Population’-level impacts
Selective breeding
Another (perhaps more complicated) system that can drive maladaptation is through selective breeding. For the vast majority of domesticated animals, selective breeding acts as a mechanism to drive the evolution of particular traits of human interest: traditionally, this might have related to correlates of productivity such as growth rate and size. Sometimes, however, this might relate to less agricultural species and traits, and is directly responsible for the plethora of breeds of dogs and cats that we see today.
When combined with a topic we touched on last week – pleiotropy – this can have some dramatic impacts on species (or, in this circumstance, breed) evolution and biology. While we might actively seek to breed in certain desirable traits (including those that we consider ‘cute’), covariation with secondary phenotypic traits can drive some maladaptive variants to become a fixture of the breed. Phenotypic traits that are directly linked to the target trait (such as how respiratory systems can be impacted by selection on snout physiology) or as a secondary impact of the non-specific nature of traditional selective breeding methods might drive maladaptation.
For example, although selective breeding for the cute short snouts and wrinkled fur of pugs also leaves the breed afflicted by heritable physiological issues associated with the lungs and breathing. This scenario applies to a number of other dog and cat breeds such as munchkins, Scottish folds and Devon Rexes.
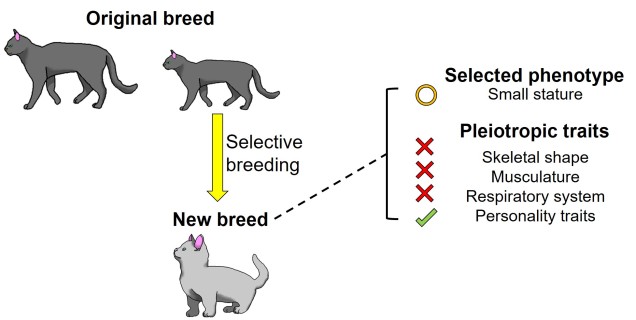
Frequency-dependent selection
Other more nuanced interactions between fitness, human impacts and genetic diversity may also drive maladaptation in populations. One way is through frequency-dependent selection, which proposes that the number of individuals (either in relative or absolute numbers) with a particular trait directly influences its overall fitness. Typically, this is used in contexts of behavioural strategies within species (e.g. ‘shy’ vs. ‘bold’ animals or male cuttlefish impersonating females) or species interactions (often related to predation strategies).
For a trait under frequency-dependent selection, fitness of the trait is directly related to the number or proportions of individuals possessing that trait. In some cases, this might be a positive relationship, with more occurrences of the trait feeding into a positive feedback loop which increases its fitness (such as in mimicry systems). In other cases, the inverse may be true, with the trait possessing higher fitness the rarer it is. Given how anthropogenic impacts can significantly impact the stability and size of populations, and that this impact may not be equally distributed across the population in question, we may (inadvertently) shifting the fitness of a trait. While empirical examples of human-driven reductions in population size leading to maladaptation don’t appear to be well-documented, there is a theoretical basis to the relationship. Additionally, other empirical studies have demonstrated this interaction under non-human-impacted scenarios, and its extension to human impacts is not a great leap.
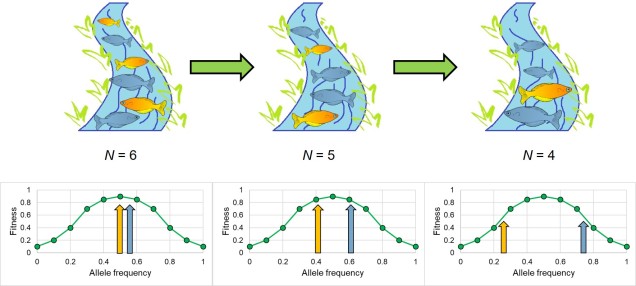
Breaking the mal
As has been reiterated here time and time again, we cannot truly appreciate the effects we have made on ecosystems across the globe. But, even with the surface that we have scratched, we can understand the significance and pervasiveness of our impacts on the evolution and adaptive capacity. If we wish to better understand of our role in driving adaptation (or not), and how we may bolster threatened species, we must also understand how we play a role in driving maladaptation in wild populations.